aBstract
The introduction of Orthogonal Polarization Spectral (OPS)
imaging and its implementation into a clinically-applicable
hand-held microscope opened the field of studying the human
microcirculation in exposed organ and tissue surfaces. Driven by
the success of OPS imaging and the drawbacks it has, we
developed a novel imaging modality for the microcirculation,
which we have termed (sidestream dark field (SDF) imaging(Renal
microcirculation,kidney
microcirculation,sublingual
microcirculation,Tongue
microcirculation,lingua
microcirculation). In
this study, we first validated SDF imaging by comparison of SDF-mediated
measurements of capillary diameters and red blood cell
velocities in the human nailfold microcirculation to
OPS¬mediated measurements. Secondly, we compared OPS and SDF
image quality, in terms of contrast and sharpness. For this
purpose, OPS and SDF images of exactly the same microcirculatory
areas were obtained sublingually. We found that SDF imaging
provides superior venular and capillary contrast compared to OPS
imaging. Thirdly, we explored the SDF imaging (Renal
microcirculation, kidney
microcirculation,sublingual
microcirculation,Tongue
microcirculation,lingua
microcirculation) capabilities with
respect to imaging individual red and white blood cells and the glycocalyx in the sublingual microcirculation. In conclusion,
the present study has introduced SDF imaging as a novel imaging
modality, incorporated in a hand-held clinically-applicable
device and validated it by quantitative comparison to OPS
imaging. It is anticipated that SDF imaging (Renal
microcirculation,kidney
microcirculation,sublingual
microcirculation,Tongue
microcirculation,lingua
microcirculation)will serve as a
novel and improved imaging modality to contribute to the
clinical assessment of the microcirculation in various clinical
scenarios and, additionally, allow more reliable application of
computer-aided image processing and analysis software for
quantification of microcirculatory alterations associated with
disease and therapy.
intrOductiOn
Up to one decade ago, direct intravital observation of the
microcirculation in humans was limited to the use of bulky
capillary microscopes, mainly applied to the nailfold capillary
bed, thus severely limiting microcirculatory investigation under
clinical conditions. The introduction of Orthogonal Polarization
Spectral (OPS) imaging(Renal
microcirculation,kidney
microcirculation,sublingual
microcirculation,Tongue
microcirculation,lingua
microcirculation) by Slaaf et al. and its implementation
into a clinically-applicable hand-held microscope opened the
field of studying the human microcirculation in exposed organ
and tissue surfaces [Slaaf et al., 1987; Groner et al., 1999].
Since then, numerous studies have been undertaken in various
clinical scenarios where cardiovascular function is at risk [Mathura
et al., 2001a;Cerný et al., 2007]. Studies have focused on the
microcirculation during disease and therapy in surgery,
emergency medicine, and intensive care medicine [Spronk et al.,
2002; Sakr et al., 2004, 2007; Ince, 2005] as well as during
such diverse conditions as cancer [Mathura et al., 2001b], wound
healing [Lindeboom et al., 2007], and infectious diseases [Dondorp
et al., 2007]. OPS imaging(Renal
microcirculation,kidney
microcirculation,sublingual
microcirculation,Tongue
microcirculation,lingua
microcirculation) has had an important clinical impact
by observation of the sublingual microcirculation during sepsis,
shock, and resuscitation [Sakr et al., 2004; De Backer et al.,
2002, 2004; Boerma et al., 2005]. Results from several medical
centers have shown that OPS observation of sublingual
microcirculatory alterations provided more sensitive information
about patient outcome from sepsis and shock than conventional
clinical parameters do. These microcirculatory alterations were
shown to be especially present in the capillaries, making their
study of particular importance [Goedhart et al., 2007; Dobbe et
al., 2007; De Backer et al., 2007].
In addition to the assessment of microvascular morphology and
perfusion, OPS imaging(Renal
microcirculation,kidney
microcirculation,sublingual
microcirculation,Tongue
microcirculation,lingua
microcirculation) can be used for identification and
measurement of the capillary glycocalyx, a physiological
compartment important for endothelial function and maintaining a
barrier function between the circulation and the tissue cells [Nieuwdorp
et al., 2005]. The endothelial glycocalyx, a negatively charged
gel-like layer, composed of proteoglycans, glycosaminoglycans,
glycoproteins and glycolipids, is considered to protect the
vascular wall by prevention of direct contact with flowing
blood. Hence, the glycocalyx contributes to vascular homeostasis
by maintaining the vascular permeability barrier, regulating the
shear stress-induced release of nitric oxide (NO) and by
inhibition of white blood cell and thrombocyte adhesion to the
vascular wall [Henry and Duling, 1999; Weinbaum et al., 2003;
Mochizuki et al., 2003; Florian et al., 2003; Thi et al., 2004].
Impaired or damaged glycocalyx is accompanied by a number
vascular wall alterations known as the earliest characteristics
of atherogenesis, a major cause of cardiovascular diseases
[Libby, 2002; Van den Berg et al., 2006; Contantinescu et al.,
2003]. Therefore, glycocalyx measurements may hold a promise as
a diagnostic tool to estimate cardiovascular risk as well as to
evaluate the impact of cardiovascular risk-lowering or even
glycocalyx-restoring therapeutic interventions Gouveneur .
Despite the major contribution OPS imaging (Renal
microcirculation,kidney
microcirculation,sublingual
microcirculation,Tongue
microcirculation,lingua
microcirculation) has made in the field
of intravital microcirculatory imaging, several shortcomings
were still present [Lindert et al., 2002; Cerný et al., 2007].
These include suboptimal imaging of the capillaries due to
motion-induced image blurring by movement of the OPS device, the
tissue, and/or flowing red blood cells. This introduces
difficulties in measuring blood flow velocities in these
vessels. Driven by the success of OPS imaging and the drawbacks
it has, we developed a novel imaging modality for the
microcirculation, which we have termed sidestream dark field (SDF)
imaging(Renal microcirculation,kidney
microcirculation,sublingual
microcirculation,Tongue
microcirculation,lingua
microcirculation) [Goedhart et al., 2007].
In this study, the first aim was to validate SDF imaging by
comparison of SDF¬mediated measurements of capillary diameters
and red blood cell velocities in the human nailfold
microcirculation to OPS-mediated measurements. The second aim of
this study was to compare OPS and SDF image quality, in terms of
contrast and sharpness. For this purpose, OPS and SDF images of
exactly the same sublingual microcirculatory areas were
obtained. The third aim was to explore the SDF imaging
(Renal microcirculation,kidney
microcirculation,sublingual
microcirculation,Tongue
microcirculation,lingua
microcirculation)capabilities with respect to imaging individual red and white
blood cells and assessment of the endothelial glycocalyx in the
sublingual microcirculation.
Ops and sdf imaging technology
For OPS imaging, a Cytocan-II backfocus type device (Cytometrics,
Philadelphia, PA) was used [Groner et al., 1999] and for SDF
imaging, a MicroScan Video Microscope (MicroVision Medical,
Amsterdam, The Netherlands) was employed.
In OPS imaging, the tissue embedding the microcirculation is
illuminated with polarized green light [Slaaf et al., 1987,
Groner et al., 1999] (Figure 1A). Backscattered (and thus
depolarized) light is projected onto a CCD camera after it
passes an analyzer, i.e., a polarizer orthogonally-oriented with
respect to the incident polarization. The light reflected by the
tissue surface, which is undepolarized, is blocked by this
analyzer. By elimination of the reflected light and imaging of
only the backscattered light, subsurface structures, such as the
microcirculation(Renal microcirculation,kidney
microcirculation,sublingual
microcirculation,Tongue
microcirculation,lingua
microcirculation), can be observed. The use of green light
ensures sufficient optical absorption by the (de)oxyhemoglobin-containing
red blood cells (red blood cells) with respect to the lack of
absorption by the tissue embedding the microcirculation,
creating contrast (i.e., red blood cells are visualized black
and tissue is visualized white/grayish).
In SDF imaging, illumination is provided by surrounding a
central light guide by concentrically placed light emitting
diodes (LEDs) to provide sidestream dark field illumination
(Figure 1B). The lens system in the core of the light guide is
optically isolated from the illuminating outer ring thus
preventing the microcirculatory image from contamination by
tissue surface reflections. Light
from the illuminating outer core of the SDF probe(Renal
microcirculation,kidney
microcirculation,sublingual
microcirculation,Tongue
microcirculation,lingua
microcirculation), which
penetrates the tissue illuminates the tissue-embedded
microcirculation by scattering. The LEDs emit at a central
wavelength of 530 nm, chosen to correspond to an isosbestic
point in the absorption spectra of deoxy-and oxyhemoglobin to
ensure optimal optical absorption by the hemoglobin in the red
blood cells, independent of its oxygenation state. This leads to
images similar to OPS images(Renal
microcirculation,kidney
microcirculation,sublingual
microcirculation,Tongue
microcirculation,lingua
microcirculation), where red blood cells are imaged
as dark moving globules against a white/grayish background. To
improve the imaging of moving structures such as flowing red
blood cells, the LEDs provide pulsed illumination in synchrony
with the CCD frame rate to perform intravital stroboscopy. This
stroboscopic imaging, (partially) prevents smearing of moving
features, such as flowing red blood cells, and motion-induced
blurring of capillaries due to the short illumination intervals.
Both the OPS and the SDF devices(Renal
microcirculation,kidney
microcirculation,sublingual
microcirculation,Tongue
microcirculation,lingua
microcirculation) are fitted with a 5¡Á objective
lens system. Illumination intensity and image focus were
modulated during imaging to obtain visually optimized images for
both techniques. Covered by a sterile disposable cap, the probes
can be placed on organ and tissue surfaces to investigate
microcirculatory morphology and perfusion under different
clinical conditions. To prevent microcirculatory perfusion
alterations by applying pressure on the imaged area, the probes
were placed onto the tissue and then gently pulled back until
contact was lost [Trzeciak et al., 2007; De Backer et al.,
2007]. Then the probes were advanced again slowly to the point
at which contact was regained and the microcirculation was in
focus of the lens systems contained in both probes.

figure 1. A) The OPS imaging
device. The green, polarized light is reflected by a half pass
mirror to provide dark field illumination. The reflected and
backscattered light travel through the hole in the mirror to the
second analyzer, termed the analyzer, with orthogonal
orientation to the first polarizer. B) The SDF imaging device.
Light emitting diodes (LEDs) provide stroboscopic sidestream
dark field illumination at 530 nm.
Compared to OPS imaging, SDF imaging(Renal
microcirculation,kidney
microcirculation,sublingual
microcirculation,Tongue
microcirculation,lingua
microcirculation) has the advantage of
low-power LED illumination, which allows battery and/or
(portable) computer operation and thereby improved clinical
applicability. For OPS imaging, relatively strong light sources
and thus mains power supply are required, since a large portion
of the illumination light is blocked by the first polarizer and
another substantial amount of light is reflected by the tissue
surface, which do not contribute to the image formation. These
high power light sources limit the portability and clinical
applicability of OPS imaging. Since SDF employs low-power LEDs
for illumination, no isolation transformers between the device
and mains power supply are required to protect current leakage
in operating rooms, intensive care units, and emergency rooms.
Furthermore, battery operation allows microcirculatory
measurements recordings to be made in conditions such as
ambulances, and emergency and combat medicine, where mains power
is not always available.
validation of sdf imaging
For the validation of SDF imaging by comparison to OPS imaging(Renal
microcirculation,kidney
microcirculation,sublingual
microcirculation,Tongue
microcirculation,lingua
microcirculation),
twenty subjects were screened for a nailfold microcirculation
that was clearly visible when applying OPS imaging. Eventually,
nine healthy non-smoking male volunteers and one healthy
non-smoking female volunteer (mean¡ÀSD age was 20¡À2 year) were
selected for this validation study. None of these subjects used
any medication and all refrained from drinking coffee at least
two hours before the measurements to ensure a stable and
uninfluenced nailfold microcirculation.
The OPS device and the SDF device(Renal
microcirculation,kidney
microcirculation,sublingual
microcirculation,Tongue
microcirculation,lingua
microcirculation) were mounted in a specially
engineered universal holder (Department of Instrumentation,
Academic Medical Center, University of Amsterdam) for accurate
positioning and stabilization of both probes and to enable quick
and easy interchanging of the devices. Video output was
visualized on a monitor and connected to a computer via a signal
converter (Canopus, ADVC110) to directly and digitally record
images onto a hard drive as DV-AVI files to enable off-line
analysis of the images for capillary diameters and red blood
cell velocities using AVA software (Automated Vascular Analysis,
Academic Medical Center, University of Amsterdam) [Dobbe et al.,
2008].
Validation of SDF imaging(Renal
microcirculation,kidney
microcirculation,sublingual
microcirculation,Tongue
microcirculation,lingua
microcirculation) was performed in analogy to our
previously published protocol, where OPS imaging was compared to intravital capillaroscopy (i.e., the gold standard for
microcirculatory imaging prior to the introduction of OPS
imaging) [Mathura et al., 2001c]. Briefly, the subjects were
seated in a comfortable and stable position with their arms
slightly bent at heart level. The fingers of the non-dominating
hand were stabilized by pushing them gently into a clay bed.
Room temperature was kept between 19 and 22 oC. By random
selection, it was decided which device (OPS or SDF) was to be
used first. Paraffin oil was applied to make the highly
scattering nailfold skin more translucent. The devices were
adjusted for optimal focus and contrast. Images were recorded
during rest, 2-min venous occlusion, and 2-min arterial
occlusion (starting 2 min after release of the venous occlusion)
to investigate the response of microcirculatory blood vessel
diameter and red blood cell flow to occlusion and release. A
cuff, which was inflated in < 5 seconds, was used for venous
(cuff pressure = 50 mmHg) and arterial (cuff pressure = 180
mmHg) occlusion. OPS and SDF images(Renal
microcirculation,kidney
microcirculation,sublingual
microcirculation,Tongue
microcirculation,lingua
microcirculation) were acquired sequentially
during each of the physiological stimuli.
From the nailfold microcirculation, four capillaries were
arbitrarily selected for further off-line analysis (Figure 2).
After stabilization (to eliminate movement artifacts) of
isolated video sequences, AVA software was used to analyze
microcirculatory blood vessel diameters and red blood cell
kinetics. Regression analysis for capillary diameters obtained
with SDF imaging and the capillary diameters obtained with OPS
imaging showed that the magnification ratio OPS:SDF equals
0.9:1.0 (slope = 0.90, R2 = 0.88). During further analysis, the
scaling factor ¡Á0.9 is applied for the SDF-mediated measurements
to correct for the magnification difference and to allow
comparison of capillary diameters and red blood cell velocities
obtained with OPS and SDF imaging.
At rest, mean¡ÀSD capillary diameters measured using OPS and SDF
imaging were 15.8¡À4.9 and 16.1¡À4.2 µm (p=0.71), respectively.
During venous occlusion, capillary diameters measured using OPS
and SDF imaging (Renal microcirculation,kidney
microcirculation,sublingual
microcirculation,Tongue
microcirculation,lingua
microcirculation)were 17.4¡À4.6 and 18.0¡À4.1 µm (p=0.51),
respectively. During arterial occlusion, capillary diameters
measured using OPS and SDF imaging were 16.1¡À4.7 and 16.0¡À3.8 µm
(p=0.93), respectively. Furthermore, Bland-Altman analysis
showed an average measurement bias of only 1.3¡À2.3 µm between
OPS and SDF imaging (plot not shown).
OPS imaging allowed the measurement of red blood cell velocities
by the use of space-time diagrams [Dobbe et al., 2008] in 36 out
of the 40 capillaries and SDF imaging allowed these measurements
in 39 out the 40 capillaries. The capillaries in which the red
blood cell velocity could not be determined were hyperperfused
resulting in velocities beyond the detection of the frame rate
of the CCD camera. At rest, mean¡ÀSD capillary red blood cell
velocities measured using OPS and SDF imaging(Renal
microcirculation,kidney
microcirculation,sublingual
microcirculation,Tongue
microcirculation,lingua
microcirculation) were 277¡À94 and
270¡À96 µm/s (p=0.60), respectively. During venous occlusion, red
blood cell velocities measured using OPS and SDF imaging were
83¡À37 and 89¡À38 µm/s (p=0.19), respectively. The red blood cell
velocities during venous occlusion were significantly lower than
during rest for both OPS and SDF imaging (p<0.01).
Microcirculatory perfusion completely stopped during arterial
occlusion as observed by both OPS as SDF imaging. Furthermore,
Bland-Altman analysis showed an average measurement bias of only
14¡À72 µm/s between OPS and SDF imaging during rest and 3¡À52 µm/s
during venous occlusion (plots not shown). Hence, SDF and OPS
imaging provide similar quantitative data on capillary diameters
and red blood cell velocities, validating the use of SDF imaging(Renal
microcirculation,kidney
microcirculation,sublingual
microcirculation,Tongue
microcirculation,lingua
microcirculation)
for studying the microcirculation.
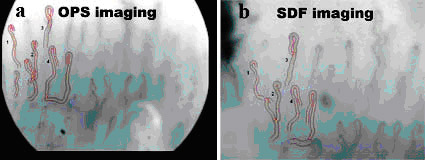
figure 2. OPS (A) and SDF
(B) image of the same nailfold capillary bed. Four capillaries
were selected for further off-line analysis of capillary
diameters and red blood cell velocities.
Ops and sdf image quality comparison
To compare image quality for OPS and SDF imaging(Renal
microcirculation,kidney
microcirculation,sublingual
microcirculation,Tongue
microcirculation,lingua
microcirculation), sublingual
images were obtained in two subjects that were trained in OPS
and SDF imaging and were able to locate the same sublingual
microcirculatory areas on command. To ease location of the
sublingual microcirculatory areas, OPS video frames were saved
and printed to serve as guides. During the sublingual
recordings, images were optimized by illumination and focus
modulation. No off-line image enhancement was performed for both
image analysis and publication.
After recording the sublingual microcirculation by OPS and SDF
imaging(Renal microcirculation,kidney
microcirculation,sublingual
microcirculation,Tongue
microcirculation,lingua
microcirculation), three microcirculatory areas were selected for image
quality analysis in terms of contrast and sharpness. In these
three areas, one video frame was isolated for both OPS and SDF.
Since the functional information in microcirculatory images lies
in the capillaries and the venules, image quality was determined
for each of these vessel types. Therefore, in each of the
sublingual microcirculatory video frames, six capillaries and
five venules were chosen to perform capillary and venular
quality analysis. To determine capillary and venular contrast
and sharpness, cross-sectional grayscale profiles (grayscale
value 0 corresponds to black and 255 corresponds to white) were
obtained using ImageJ (developed at the US National Institutes
of Health). The contrast was defined as the
absolute difference between the minimum value within the vessel
and the maximum value (average of two sides of the vessel). The
sharpness was defined as the angle of the grayscale profile at
the vessel wall (average of two sides of the vessel).
For OPS and SDF imaging(Renal
microcirculation,kidney
microcirculation,sublingual
microcirculation,Tongue
microcirculation,lingua
microcirculation), similar capillary (77¡À7 and 79¡À6;
p=0.37) and venular sharpness (74¡À8 and 72¡À7; p=0.23) were
found. Capillary (14¡À8 and 23¡À12; p<0.01) and venular (47¡À23 and
56¡À25; p=0.05) contrast, however, were found to be higher in SDF
images compared to in OPS images. In Figures 3A and 3B, the
capillary and venular quality for OPS and SDF imaging are
illustrated. Figure 3A clearly shows that capillaries have
higher contrast when using SDF imaging compared to when using
OPS imaging. Venular contrast and sharpness is approximately
equal for both techniques as shown in Figure 3B. Additionally,
the Figures depict the magnification difference between the OPS
and SDF device (Renal microcirculation,kidney
microcirculation,sublingual
microcirculation,Tongue
microcirculation,lingua
microcirculation)(scale bars Figures 3A and 3B).
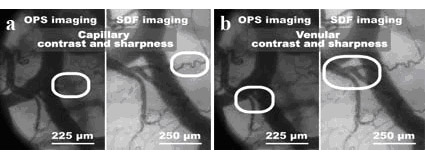
figure 3. A) Capillary
contrast and sharpness. B) Venular contrast and sharpness. The
scale bars indicate the magnification difference between the OPS
and SDF device.
imaging individual red and white blood cells Using SDF imaging(Renal
microcirculation,kidney
microcirculation,sublingual
microcirculation,Tongue
microcirculation,lingua
microcirculation),
individual red and white blood cells can be observed. This is
exemplified in Figures 4 and 5. Figure 4 shows individual red
blood cells, separated by plasma gaps, flowing trough a
capillary loop. In Figure 5, most white blood cells travel from
the vertical capillary via the T-junction to the right capillary
(Figure 5B, 5C, and 5D). As the capillary increases in diameter,
the white blood cells start rolling till they reach the
capillary-venule junction. Once arrived in the venule some white
blood cells remain rolling against the venular wall and others
are taken up by the blood flow and slowly flow down-stream.
imaging the endothelial glycocalyx Provided that white blood
cells are sufficiently stiff to (temporarily) damage the
endothelial glycocalyx during their passage in small capillaries
(i.e., < 10 µm), while the glycocalyx in turn is stiff enough to
deform red blood cells, the red blood cell column width in
before and after white blood cell passage can be used to
estimate glycocalyx thickness. Hence, using SDF imaging of the
sublingual microcirculation(Renal
microcirculation,kidney
microcirculation,Tongue
microcirculation,lingua
microcirculation), estimations of individual capillary glycocalyx dimensions could be obtained. For glycocalyx
measurements, it is first important to distinct white blood
cells from plasma gaps, which can be done by following the white
blood cell/plasma gap to a capillary-venule junction (Figures
6A-6D). At this junction, white blood cells will tend to roll
against the venular wall, while plasma gaps will dissolve in the
larger blood stream (Figure 6D). Once an image is captured from
before
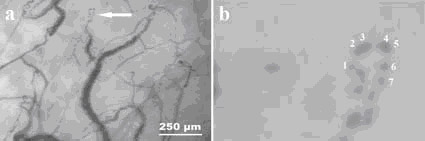
figure 4. Individual red
blood cells (numbered from 1 to 7) and plasma gaps flowing
through a capillary can be observed in normal view (A) and in
the zoomed view (B).
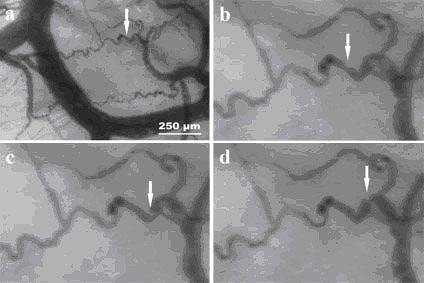
figure 5. SDF imaging
enables white blood cell visualization in normal view (A) and in
zoomed view (B, C, and D). The red arrows indicate a rolling
white blood cell at t=0 ms (A and B), t=400 ms (C), and t=800 ms
(D). and after the white blood cell passage, an estimation of
the glycocalyx thickness can be made, by subtracting the initial
red blood cell column diameter from the diameter after white
blood cell passage (Figures 6E and 6F).
discussiOn and cOnclusiOns
This study introduced a novel optical technique for clinical
observation and assessment of the microcirculation, termed sidestream dark field (SDF) imaging, and validated it by
quantitative comparison to OPS imaging. Results showed that OPS
and SDF imaging provided similar values for capillary diameters
and red
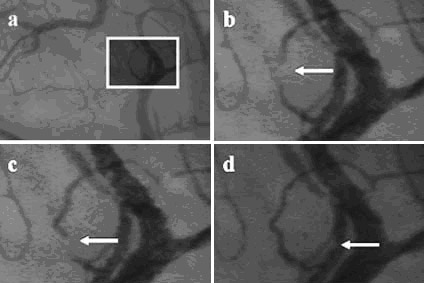
figure 6. A)
Sublingually-acquired microcirculatory image using SDF imaging.
The white square indicates the region of interest, which is
enlarged in the following panels. B and C) White blood cell
flowing through a capillary (indicated with arrows). D) Same
white blood cell, rolling against venular wall. E) Enlarged view
of the capillary without (left side) and with (right side) the
white blood cell. F) Same as panel (E), with enhanced contrast
for more clear observation of the widening of the red blood cell
column after the white blood cell passage (indicated with
arrows).
blood cell velocities in the human nailfold microcirculation.
These basic findings validate the use of SDF imaging for
clinical measurement of microcirculatory vessel diameters and
red blood cell velocity measurements. SDF imaging, moreover,
provided significantly higher image quality with more detail and
higher capillary and venular contrast and enabled imaging of
individual red and white blood cells and measurement of the
endothelial glycocalyx thickness.
Increased microvascular quality and observability of granular
structures probably originates from the stroboscopic
illumination, which prevents smearing of moving features such as
red blood cell columns in capillaries and venules. Stroboscopic
imaging also reduces image blurring due to movement of the
device and/or the tissue. An additional contributing factor to
the superior quality of SDF imaging is the shallower focusing
depth of the SDF device with respect to
the focus of the OPS device(Renal
microcirculation,kidney
microcirculation,sublingual
microcirculation,Tongue
microcirculation,lingua
microcirculation). In OPS imaging, underlying vascular
(and thus light absorbing) structures (partially) darken the
image, lowering image contrast and quality. In SDF imaging
however, these underlying structures do not interfere, due to
the shallow imaging depth of the SDF device, and therefore
provide clear images of the superficial microcirculatory
network.
However, although SDF imaging(Renal
microcirculation,kidney
microcirculation,sublingual
microcirculation,Tongue
microcirculation,lingua
microcirculation) was shown to be superior to OPS
imaging, it still suffers from some shortcomings. In the current SDF device, the detectable red blood cell velocity is physically
limited (at approximately 1 mm/s) by the length of the observed
vessels in combination with the 25 fps (PAL) or 30 fps (NTSC)
acquisition rates. Hence, future improvement of microcirculatory
imaging will be made by incorporation of more advanced camera
technology in terms of resolution and frame rate, which will
enable red blood cell velocity measurements in high flow vessels
and more accurate vessel geometry determination. This, in
conjunction with completely automated software, with (new)
microcirculatory scoring systems implemented, will lead to
faster and more exact determination of microcirculatory
functioning in clinical and experimental settings.
Another point of concern with SDF imaging(Renal
microcirculation,kidney
microcirculation,sublingual
microcirculation,Tongue
microcirculation,lingua
microcirculation) is the
pressure-induced microcirculatory alterations by application of
the SDF probe onto organ and tissue surfaces. These
pressure-induced effects occur with other surface flow/perfusion
measurements, such as OPS imaging and laser Doppler velocimetry,
as well and might lead to false interpretation of the actual
microcirculatory perfusion. To prevent the microcirculatory
measurements from this pressure artifact during OPS imaging(Renal
microcirculation,kidney
microcirculation,sublingual
microcirculation,Tongue
microcirculation,lingua
microcirculation), Lindert et al. engineered an extending click-on ring, which was
placed around the OPS probe. By applying suction via holes in
the ring using a vacuum pump, the tissue in the center, that was
imaged through the OPS probe, was inhibited from moving and the
pressure on the imaged vasculature was reduced [Lindert et al.,
2002]. However, in order to objectify the assessment of the
microcirculatory perfusion, the pressure artifact should be
characterized, i.e., the distortion of microcirculatory
perfusion should be measured as a function of the applied
pressure.
In conclusion, the present study has introduced SDF imaging(Renal
microcirculation,kidney
microcirculation,sublingual
microcirculation,Tongue
microcirculation,lingua
microcirculation) as a
novel imaging modality, incorporated in a hand-held
clinically-applicable device. SDF imaging was validated by
quantitative comparison to OPS imaging(Renal
microcirculation,kidney
microcirculation,sublingual
microcirculation,Tongue
microcirculation,lingua
microcirculation). It is anticipated that SDF imaging will serve as a novel and improved imaging modality
to contribute to the clinical assessment of the microcirculation
in various clinical scenarios and, additionally, allow more
reliable application of computer-aided image processing and
analysis software for quantification of microcirculatory
alterations associated with disease and therapy. |